1.IntroductionThe skin, composed of the epidermis, dermis, and subcutaneous tissues, is the body’s largest organ.1 The outermost stratum corneum comprises multiple layers of inactive keratinocytes held together by a lipid-rich matrix. These cells overlap to effectively prevent intravasation of foreign substances and extravasation of body fluids. The remainder of the epidermis consists of the hyaline, granular, spinous, and basal layers. The dermis contains abundant collagen and elastic fibers that provide toughness and elasticity. It is also rich in blood vessels, lymphatics, and nerves that regulate body temperature, sense external stimuli, and provide immune defense. The skin thus plays a crucial role in maintaining the body’s normal physiological state. When lesions occur within the body, the skin may be affected, resulting in cutaneous structural and functional abnormalities that manifest as various diseases.2,3 Optical imaging techniques have been widely applied in skin imaging due to their noninvasiveness, high resolution, and high contrast. However, skin is a turbid medium with a complex composition and varying refractive indices,4,5 which limits the optical imaging quality. In 1997, Tuchin’s group proposed a tissue optical clearing technique using chemical agents to render tissues transparent.6 This technique was quickly applied to skin and garnered significant attention. Initially, researchers explored rendering skin transparent ex vivo,7 allowing for the acquisition of cutaneous structural information but not permiting observation of live dynamics. Then, researchers began to apply the optical clearing technique to skin in vivo by directly injecting optical clearing agents (OCAs) into the dermis to improve skin transmittance. However, this protocol was found to adversely affect skin vasculature8 and even cause skin necrosis in some cases.9 In 2010, Zhu’s group successfully achieved in vivo skin optical clearing imaging by topically applying polyethylene glycol (PEG)-400 and thiazone and observing the distribution of blood flow in rat dermis with high resolution using laser speckle contrast imaging (LSCI).10 Since then, more and more in vivo skin OCAs have been discovered. Furthermore, researchers attempted to move the optical clearing method to human skin.11–13 In 2016, Leahy’s group successfully improved the in vivo OCT imaging quality in human forearm skin using fructose gel.14 The applications and potential of in vivo skin optical clearing technique on human subjects were explored, and its significance in clinical diagnosis and treatment was highlighted in the book published in 2022.15 The in vivo skin optical clearing technique enabled various optical imaging techniques to obtain richer and finer skin-related information and has already been applied in the basic and clinical studies, such as identifying skin lesions,16 detecting disease-induced cutaneous dysfunction,17 and localizing transdermally delivered drugs.18 With the aid of skin optical clearing, safe, high-efficiency, and accurate light-induced therapy can be achieved for purposes, such as oncotherapy,19 tattoo removal,20 and photocoagulation.21,22 This review first examines the development of skin optical clearing methods before detailing the important role of in vivo skin optical clearing techniques in enhancing optical imaging performance on skin, facilitating disease study and laser treatment. 2.In Vivo Skin Optical Clearing MethodsThe skin optical clearing technique has rapidly advanced since its proposal, benefiting from ongoing progress in screening OCAs and deepening understanding of underlying mechanisms. Initially, researchers speculated that OCAs with high osmotic pressure and refractive index could cause an outflow of low-refractive-index water in tissue and penetrate the tissue to partially replace interstitial fluid, increasing the refractive index of the liquid matrix and improving refractive index matching between the liquid matrix and skin scatterers. However, subsequent studies revealed that the optical clearing potential of agents was not necessarily correlated with their refractive index or osmotic pressure but was more correlated to their molecular structure.23,24 Molecules with hydroxyl groups can compete for and occupy key sites on dermal collagen that form hydrogen bonds with water molecules, disrupting the surrounding hydration layer, releasing bound water, and destabilizing the triple helix structure of collagen.25 Agents with more hydroxyl groups were thus preferred.26 Later, agents with a propensity to form higher hydrogen bond bridge types were predicted to have better optical clearing effects.27 Higher bridge types span further across the collagen surface and more severely disrupt collagen–collagen and collagen–water interactions. The molecular coverage of agents on key collagen sites is related not only to the span of hydrogen bridges but also to their molecular sizes. Considering these two factors, a more promising indicator, the molecular effective coverage surface area, was proposed.28 These indicators increasingly efficiently predicted the optical clearing potential of chemical agents, enabling the identification of sugars and alcohols with high optical clearing potential.28–30 In theory, OCAs can render skin transparent through a combined effect of dehydration, collagen dissociation, and refractive index matching, as shown in Fig. 1(a). However, in realistic in vivo applications, the optical clearing effect of agents is sometimes unsatisfactory due to the dense stratum corneum seriously hindering agent penetration into the skin. To overcome this barrier, researchers have proposed various physical methods to promote the penetration of OCAs, including laser irradiation,13 fractional laser microablation,34 adhesive tape stripping,35 sandpaper rubbing,36 sonophoresis,37 microneedle,38 flashlight irradiation,39 and more. These methods enhance the OCA penetration by directly removing the stratum corneum and part of the living epidermis, creating microchannels in the skin or generating heat effects to locally disrupt the stratum corneum. Some chemical agents have also been shown to improve the in vivo skin optical clearing efficiency to varying degrees, including ethanol,31 dimethyl sulfoxide (DMSO),40 propylene glycol (PG),41,42 salicylic acid,43 liquid paraffin,11,44 hyaluronic acid,45 oleic acid,18,37 and so on. As shown in Fig. 1(b), by combining OCAs with penetration-enhancing methods, in vivo skin optical clearing methods applicable to different body parts have been successfully developed based on their physiological characteristics.31–33 Fig. 1Overview of in vivo skin optical clearing technique. (a) Schematic diagram of in vivo skin optical clearing technique. The figure was created using BioRender. (b) Typical photographs of skin in different body parts before and after in vivo skin optical clearing.31–33 ![]() For in vivo skin optical clearing, the biocompatibility of agents is also a major concern. Some clinically used contrast agents with a high refractive index have been proposed as novel OCAs, such as magnetic resonance imaging contrast agent gadobutrol (Gadovist®),46,47 gadopentetate dimeglumine (Magnevist®),46 gadoterate meglumine (Dotarem®)46 and iso-osmolar x-ray contrast agent iodixanol (Visipaque™),46 and iohexol (Omnipaque™).48,49 Their ex vivo and (or) in vivo applications exhibited a certain optical clearing effect with minor tissue deformation, and no tissue inflammation or hyperemia was observed. It is expected that these contrast agents have high potential for clinical applications. In the field of optical imaging, diagnosis, and treatment of skin, researchers typically use visible-NIR light and closely monitor changes in tissue reflectance or transmittance in the visible-NIR range when evaluating the effect of skin optical clearing methods.50 However, other wavelength bands are also critical for skin-related studies. For instance, moderate ultraviolet exposure can promote the production of vitamin D in the body51 and treat skin diseases such as atopic dermatitis and psoriasis.52,53 Terahertz wave can be used to diagnose skin cancer and determine the extent of skin burns based on skin hydration status.54 Therefore, researchers have also investigated the applicability of skin OCAs in a wider spectral range. It was verified that ex vivo treatment with glycerol could increase the transmittance of colorectal muscle samples at the ultraviolet band (200 to 400 nm) to varying degrees.55 The improvement of two bands with central wavelengths located at 230 and 300 nm was particularly significant. In addition, PG and/or glycerol were found to create optical clearing windows in the UV range of gingival mucosa.56–58 For the THz band, the strong absorption of water is a critical factor limiting its propagation in biological tissues. Decreased tissue’s water concentration induced by optical clearing is expected to increase THz transparency.59 To identify suitable OCAs for THz applications, Musina et al. comprehensively compared THz-wave absorption and diffusion coefficients in rat brain for multiple agents, including glycerol, PG, DMSO, sucrose, glucose, fructose, dextran 40 and 70, and PEG with different molecular weights. Glycerol was found to be the optimal choice due to its combination of low THz-wave absorption coefficient and high diffusion coefficient.60 The ability of glycerol to improve THz imaging was further confirmed in ex vivo porcine skin.61 The wider spectral application range will greatly broaden the application scenarios of the in vivo skin optical clearing technique. 3.In Vivo Skin Optical Clearing for Improvement of Imaging PerformanceOptical imaging techniques have been widely used to obtain structural, component, and functional information about the skin. However, due to the turbid nature of skin, the imaging ability of optical imaging techniques is limited, which can result in missing useful information. To overcome this limitation, researchers have applied the in vivo skin optical clearing technique to improve the imaging quality of various optical imaging techniques and explore richer and finer skin-related information. With the assistance of skin optical clearing, LSCI and hyperspectral imaging (HSI) could map cutaneous blood flow and blood oxygen saturation in the mice skin, as shown in Figs. 2(a) and 2(b), enabling the quantitative assessment of hemodynamic responses to stimuli with the single-vessel resolution and improved velocity sensitivity.62,65 Optical coherent tomography (OCT) and its extension could visualize cutaneous layered structures66,67 and detect three-dimensional vascular networks in mice and human skin,14,35,63 as shown in Fig. 2(c).35 Furthermore, photoacoustic imaging (PAI) could provide deeper and richer vasculature in mice limbs when glycerol was mixed with ultrasound gel for acoustic coupling,64 as shown in Fig. 2(d). Skin optical clearing also improved Raman spectroscopy to detect stronger and deeper Raman peaks of skin68 and assisted surface-enhanced Raman scattering imaging in identifying the shape and boundary of the tumor phantom more accurately,69,70 which would help determine the location of tumors and the presence of metastases. In addition, skin optical clearing increased detection sensitivity of circulating cells by flow cytometry71,72 and improved the signal intensity and imaging depth of laser confocal microscopy when detecting monocytes in mice footpads,31 as shown in Fig. 2(e). This advancement would be beneficial for tracking cellular activity deep within the skin. Fig. 2Overview of improvement of optical imaging with in vivo skin optical clearing. (a) Blood flow images of mice dorsal skin acquired with LSCI.62 (b) Blood oxygen saturation images of mice dorsal skin acquired with HSI.62 (c) Projection of angiographic images of mice dorsal skin acquired with OCT.63 (d) Angiographic images of mice limb acquired with PAI.64 (e) Maximum intensity projection of fluorescence images in mice footpad skin acquired with laser scanning confocal microscopy.31 ![]() In vivo skin optical clearing technique assists optical imaging to obtain greater imaging depth, higher contrast, resolution, and detection sensitivity, but it should be noted that optical imaging techniques are selective to OCAs. Sometimes OCAs can even have adverse effects on imaging quality. For example, the in vivo application of PEG-400 and thiazone on rat dorsal skin could greatly enhance PA amplitude and improve the image quality of deep vessels, whereas DMSO would cause a decrease in PA amplitude, making it a bad choice for improving PAI.73 In addition, it is equally important for researchers to focus on the dynamic changes of the optical clearing effect after the application of OCAs. When applied in vivo on the skin, agents will penetrate into the skin and cause dehydration. In turn, the skin will react to these changes by rehydration, metabolism of agents, and so on, leading to a reduction in the optical clearing effect. For example, when human palm skin was treated with glucose in vivo, the improvement in imaging depth of OCT reached a peak after 15 min and then gradually declined, which indicated that about 15 min after optical clearing was the optimal time to perform imaging in this case.12 4.In Vivo Skin Optical Clearing for Aiding Disease StudySkin is a common target for both basic and clinical studies. On the one hand, the skin is exposed to the environment and serves as the first line of physiological defense against physical, chemical, and microbial threats, making it particularly susceptible to damage and a variety of associated diseases have not yet been fully understood. On the other hand, there is a close link between the effects of some diseases on the skin and internal organs, such that the skin might be implicated when other organs become diseased. Researchers have applied optical imaging to perform in vivo studies of skin lesions,74 but acquired limited information. By incorporating in vivo skin optical clearing technique, researchers can improve skin transparency and gain better insight into the structures, functions, and components of the skin. This improvement would significantly enhance the understanding of skin conditions under different diseases and underlying mechanisms of skin lesions. With the assistance of skin optical clearing imaging, the vasculature of facial skin in patients with port-wine stains (PWS) was clearly visualized, and structural parameters of blood vessels at different depths were quantitively evaluated,16 as shown in Fig. 3. The vessel density in the superficial layer () of the lesion area was lower than that of the contralateral normal skin, while the vessel diameter was similar. However, it was found that the diameter and density of deep blood vessels () were significantly higher than that of the contralateral normal skin. These parameters not only reveal the effects of PWS on blood vessels but also help doctors plan treatment. For example, in the laser treatment of PWS based on selective photothermolysis,75 setting different laser pulse widths and energies according to the size and involved depth of malformed vessels in the lesion area may achieve better treatment outcomes. Fig. 3Representative results of a study on PWS assisted with in vivo skin optical clearing imaging.16 (a) Photographs of PWS patients. The observed lesions of case 1, case 2, and case 3 were located on the cheek, the tempus, and around the lips, respectively. Red boxes represent lesion areas imaged by OCT angiography. Vasculatures of contralateral normal areas (b) and lesion areas (c) of PWS patients captured by OCT angiography after skin optical clearing. ![]() In vivo skin optical clearing technique allows continuous monitoring of the dynamic changes in skin and the impact of diseases on it. In the Henoch–Schonlein purpura rat model, changes in the morphology of blood vessels and deposition of immune complexes IgA were recorded in vivo during the acute development of skin purpura.76 It was found that vascular lesion appeared immediately after the model was established, while the deposition of IgA peaked after 12 h. It was deducted that the vascular lesion enhanced IgA deposition. Similarly, in the psoriatic mice model, cytokines in blood vessels were traced during the development of psoriasis.77 A noticeable delay between the emergence of IL-23 and IL-17 was observed, which might help explain the complex interplay among cytokines related to psoriasis. Diabetes mellitus is a kind of chronic metabolic disease. Long-term hyperglycemia can cause damage to different organs and tissues78 and further induce various complications. Some studies on diabetes-induced skin dysfunction have been conducted with the help of skin optical clearing techniques. To test vasomotor function, the hemodynamic response of mice skin induced by the vasoconstrictor noradrenaline was monitored in vivo by LSCI and HSI. The single-vessel resolution achieved by skin optical clearing allowed researchers to analyze abnormal changes in the arteries and veins separately. For normal mice, norepinephrine caused arterial and venous blood flow and blood oxygen levels to decrease within minutes, then return to initial levels or slightly increase within the monitoring time. However, in the mice model of type 1 diabetes (T1D), blood flow recovery became increasingly difficult as T1D progressed, and the decrease of arterial blood oxygen induced by noradrenaline was weakened, as shown in Fig. 4(a). It suggested that diabetes induced vasomotor dysfunction.62 Furthermore, the effects of diabetes on cerebral and cutaneous hemodynamic responses to drug stimulation were compared.80 Both cerebral and cutaneous blood oxygen responses became abnormal after 1 week, indicating that the cutaneous blood oxygen response has the potential to serve as a good assessment indicator of cerebrovascular dysfunction in the early stage of diabetes. In addition, increased vascular permeability caused by diabetes was directly observed by visualizing the distribution of intravenously injected Evans blue dye in the cutaneous vascular and extravascular regions through a skin optical clearing window. The effect of diabetes on immune function was also studied. Delayed-type hypersensitivity (DTH) was elicited in mice footpads.79 Compared with non-T1D mice, the increased infiltration and recruitment of monocytes/macrophages after DTH was found in diabetic mice, as shown in Fig. 4(b), but their motility was significantly attenuated, indicating that the immune function of monocytes/macrophages became abnormal. These tendencies were further potentiated with the progression of diabetes. The improvement of the optical imaging depth with in vivo skin optical clearing allowed the comparison of motion behaviors of monocytes/macrophages at different depths around the inflammatory foci. For non-T1D mice, the migration displacement of monocytes/macrophages of the deep dermis was significantly larger than that of the superficial layer in the early stage of DTH (DTH-4 h), but an opposite tendency at the late stage (DTH-72 h), indicating that the cutaneous immune response at different depths was indeed different. Diabetes-induced skin structural change has also been reported. When the tissue structure changes, it will affect the diffusion of agents in the tissue. Therefore, in addition to direct visualization, it is also possible to indirectly diagnose tissue structural changes by measuring the diffusion of agents in the tissues. A comparative study of the diffusion of glucose in ex vivo skin samples of normal or alloxan-induced diabetic rats was conducted by measuring dynamic changes of collimated transmittance and geometric parameters (weight, thickness, and area) of samples in the optical clearing process.81 It was found that glucose diffusivity was twofold slower for diabetic skin. A similar conclusion was drawn by measuring the diffusion of glycerol in the normal/diabetic skin.82 Therefore, monitoring the diffusion of OCAs in the tissues can be used as a reference to distinguish between normal and diseased tissues. Fig. 4Representative results of basic studies on diabetic-induced cutaneous vascular and immune dysfunction assisted with in vivo skin optical clearing imaging. (a) Dynamic responses of blood flow maps and oxygen saturation maps to noradrenaline in non-T1D mice and diabetic mice at different stages (T1D-1 w, T1D-2 w, and T1D-4 w) monitored by LSCI and HSI through an optical clearing skin window.62 (b) Recruitment of monocytes/macrophages in mice footpads at different time points (DTH-4 h, DTH-24 h, DTH-48 h, and DTH-72 h) on non-T1D mice and diabetic mice at different stages (T1D-1 w, T1D-2 w, and T1D-4 w) acquired by confocal imaging. White arrows indicate the formed cellular clusters.79 ![]() The skin optical clearing technique is not only useful for studying skin lesions but also for detecting substances in the skin. This technique has been shown to enable more complete and deep localization of particles in the skin,18 which could be valuable for evaluating the penetration effect of novel carrier particles developed for transdermal drug delivery or obtaining pharmacokinetic information about drugs in the skin. In addition, in vivo visualization of subcutaneous upconversion nanoparticle microarrays was achieved with high spatial resolution using fluorescence imaging when the skin was cleared. It methodologically demonstrated the potential of skin optical clearing-assisted fluorescence imaging with PDMS microarrays spotted with specific probes for high-throughput detection of disease-related biomarkers in the tissue interstitial fluid.83 5.In Vivo Skin Optical Clearing for Enhancing Light-Induced TherapyWith the advent and rapid development of laser technology, it is becoming increasingly common to use lasers for medical treatment. However, to achieve optimal therapeutic outcomes, the selection of lasers is crucial. Factors, such as laser wavelength, pulse width, and energy, must be carefully considered in accordance with the specific disease, condition, and lesion location.84,85 It is also important to overcome the challenges posed by the tissue above the target area. Scattering and absorption will reduce the luminous flux reaching the target and prevent the light from focusing on the target. While increasing laser power may improve treatment outcomes to a certain extent, it can also lead to greater damage to the surrounding tissues. Simulations have demonstrated that increased skin transparency played a critical role in enhancing the luminous flux reaching the target layer or increasing the proportion of photons absorbed by the target layer, potentially improving treatment efficiency.20,86 Thereby, researchers have tried to introduce the skin optical clearing technique to enhance efficacy of light-induced therapy. Skin optical clearing has proven to be a valuable technique in photocoagulation. In the treatment of PWS, multi-pulse Nd: YAG laser was deduced to be powerful by coagulating diseased vessels.87,88 When the OCA was applied to the subcutaneous side of a rat dorsal skin chamber for 10 min, the number of pulses required to achieve the desired treatment endpoint (i.e., thread-like constriction of target blood vessels) was reduced by compared with treatments without optical clearing,21 as shown in Fig. 5(a). In the photocoagulation of vas deferens for sterilization, an OCA was non-invasively delivered into the scrotal skin around the laser irradiation site, and after 30 min, skin transparency was improved by 26%, which resulted in a reduction in the average power required for laser thermal coagulation of the vas deferens, from 9.2 to 7.0 W.89 Fig. 5Overview of effects of in vivo skin optical clearing on light-induced therapy. (a) Thermal response of blood vessels without or with optical clearing when irradiated with different numbers of laser pulses.21 (b) Fluorescence images of TUNEL- and Ki67-labeled tumor slices obtained 14 days after synergistic treatment of photothermo-chemotherapy in a deep-sited tumor model without or with optical clearing.19 (c) Photographs of three pigmentation areas on rat dorsal skin before and after different treatments. Area 1: laser irradiation after skin optical clearing; area 2: only laser irradiation; area 3: without treatment.20 ![]() Combined with skin optical clearing, light-induced therapy, including photodynamic therapy (PDT) and photothermal therapy (PTT), was used to treat deep-sited tumors. An in vivo experiment demonstrated that preliminary treatment of OCAs and low-intensity laser irradiation on the skin could reduce skin thermal damage during PTT of subcutaneous tumors.90 It not only indicated that light-induced therapy with the aid of skin optical clearing technique produced lower side effects, but also implied that more photons would reach the target tumor, which might lead to higher treatment efficiency. In a pigmented melanoma mouse model, PDT was performed using two commercially available photosensitizers, i.e., photodithazine (PDZ) and Visudyne (VIS).91 Dynamic changes in tumor volume were measured after PDT, and it was found that the tumor treated with PDZ-based PDT (PDZ-PDT) kept growing, similar to the untreated group. However, VIS-based PDT (VIS-PDT) and PDT based on both photosensitizers (PDZ-VIS-PDT) could reduce the tumor growth rate, but the tumor volume still increased slowly. When PDT was combined with the skin optical clearing technique, no obvious tumor growth was observed within 8 days after PDZ-PDT, and tumors treated with VIS-PDT and PDZ-VIS-PDT gradually regressed. In another study, a self-developed nano-platform DOX@PGNCs (that is, doxorubicin-loaded gold nanocages) was used to treat tumors with the synergistic effect of photothermo-chemotherapy.19 The synergistic therapy was effective in inhibiting growth of superficial tumors, but the effect was greatly weakened in tumors covered by ex vivo porcine skin. However, the application of skin OCAs brought a series of positive effects, including increased photothermal conversion efficiency of GNCs, uptake of DOX@PGNCs by tumor cells, and the release of doxorubicin, which promoted tumor cell apoptosis and inhibited tumor cell proliferation to a greater extent, as shown in Fig. 5(b). This greatly slowed the tumor growth rate. In the study of Chu et al., PTT triggered by NIR-II light was performed to enhance the effective depth of in vivo cancer therapy, thus inhibiting tumor growth. On this basis, the skin optical clearing technique was further introduced to the therapy, and the complete tumor ablation was well achieved without any reoccurrence.92 In addition, skin optical clearing has also been shown to have a positive effect on tattoo removal. When pigmented skin was pretreated with optical clearing, the clearance rate of pigment could reach up to 82% after laser irradiation, compared with only 55% clearance in the pigmented area treated with laser irradiation alone,20 as shown in Fig. 5(c). These results suggested that skin optical clearing increased the efficiency of laser tattoo removal by 1.5 times. Therefore, the application of skin optical clearing can improve treatment efficiency or decrease light dosage, which will greatly enhance the clinical translational potential of light-induced therapy. 6.Conclusion and ProspectResearchers have worked to overcome challenges posed by the strong scattering property of tissues in optical techniques, making significant improvements in optical systems93,94 and image processing.95,96 In recent decades, the novel skin optical clearing technique has been proposed to address this issue at its root by matching the refractive index of skin components. This review primarily covers the development of skin optical clearing methods and their in vivo applications in enhancing optical imaging quality, facilitating disease study, and improving the efficiency of laser treatment. While in vivo skin optical clearing has made significant contributions to basic research, its clinical applications remain limited. This is due to the fact that although efficient in vivo skin optical windows have been successfully established in experimental animals, human skin is thicker and has a stronger barrier function in the stratum corneum, making in vivo optical clearing of human skin less effective than in experimental animals. In the near future, developing high-efficiency and safe in vivo human skin optical clearing methods will be of great significance. At the same time, considering differences in human age, skin color, and body parts, niche-targeting skin optical clearing methods should be established for clinical applications, such as pediatric injection, dermoscopy, and ultrasonography. AcknowledgmentsThis work was supported by the National Natural Science Foundation of China (NSFC) (Grant No. 61860206009). ReferencesZ. Zaidi, S. W. Lanigan,
“Skin: structure and function,”
Dermatology in Clinical Practice, 1
–15 Springer, London
(2010). Google Scholar
M. Hellmann, M. Roustit and J.-L. Cracowski,
“Skin microvascular endothelial function as a biomarker in cardiovascular diseases?,”
Pharmacol. Rep., 67
(4), 803
–810 https://doi.org/10.1016/j.pharep.2015.05.008
(2015).
Google Scholar
E. Makrantonaki et al.,
“Diabetes mellitus and the skin,”
Rev. Endocr. Metab. Disord., 17
(3), 269
–282 https://doi.org/10.1007/s11154-016-9373-0
(2016).
Google Scholar
R. Drezek, A. Dunn and R. Richards-Kortum,
“Light scattering from cells: finite-difference time-domain simulations and goniometric measurements,”
Appl. Opt., 38
(16), 3651
–3661 https://doi.org/10.1364/AO.38.003651 APOPAI 0003-6935
(1999).
Google Scholar
V. V. Tuchin,
“Optical clearing of tissues and blood using the immersion method,”
J. Phys. D Appl. Phys., 38
(15), 2497
–2518 https://doi.org/10.1088/0022-3727/38/15/001
(2005).
Google Scholar
V. V. Tuchin et al.,
“Light propagation in tissues with controlled optical properties,”
J. Biomed. Opt., 2
(4), 401
–417 https://doi.org/10.1117/12.281502 JBOPFO 1083-3668
(1997).
Google Scholar
G. Vargas et al.,
“Use of osmotically active agents to alter optical properties of tissue: effects on the detected fluorescence signal measured through skin,”
Lasers Surg. Med., 29
(3), 213
–220 https://doi.org/10.1002/lsm.1110 LSMEDI 0196-8092
(2001).
Google Scholar
E. I. Galanzha et al.,
“Skin backreflectance and microvascular system functioning at the action of osmotic agents,”
J. Phys. D Appl. Phys., 36
(14), 1739
–1746 https://doi.org/10.1088/0022-3727/36/14/313
(2003).
Google Scholar
X. Wen et al.,
“In vivo skin optical clearing by glycerol solutions: mechanism,”
J. Biophotonics, 3
(1-2), 44
–52 https://doi.org/10.1002/jbio.200910080
(2010).
Google Scholar
D. Zhu et al.,
“Imaging dermal blood flow through the intact rat skin with an optical clearing method,”
J. Biomed. Opt., 15
(2), 026008 https://doi.org/10.1117/1.3369739 JBOPFO 1083-3668
(2010).
Google Scholar
K. Chen, Y. Liang and Y. Zhang,
“Study on reflection of human skin with liquid paraffin as the penetration enhancer by spectroscopy,”
J. Biomed. Opt., 18
(10), 105001 https://doi.org/10.1117/1.JBO.18.10.105001 JBOPFO 1083-3668
(2013).
Google Scholar
M. Kuck et al.,
“In vivo enhancement of imaging depth for optical coherence tomography by eudermic agents on ridged and meshed human skin,”
Laser Phys. Lett., 11
(3), 035602 https://doi.org/10.1088/1612-2011/11/3/035602 1612-2011
(2014).
Google Scholar
Z. Zhan, H. Wei and Y. Jin,
“Assessment of laser-induced acceleration effects in optical clearing of in vivo human skin by optical coherence tomography,”
Laser Phys., 25
(2), 025603 https://doi.org/10.1088/1054-660X/25/2/025603 LAPHEJ 1054-660X
(2015).
Google Scholar
J. Enfield et al.,
“Enhanced in vivo visualization of the microcirculation by topical application of fructose solution confirmed with correlation mapping optical coherence tomography,”
J. Biomed. Opt., 21
(8), 081212 https://doi.org/10.1117/1.JBO.21.8.081212 JBOPFO 1083-3668
(2016).
Google Scholar
E. A. Genina et al.,
“In vivo skin optical clearing in humans,”
Handbook of Tissue Optical Clearing: New Prospects in Optical Imaging, 369
–382 CRC Press, Boca Raton
(2022). Google Scholar
Y. Liu et al.,
“Penetration-enhanced optical coherence tomography angiography with optical clearing agent for clinical evaluation of human skin,”
Photodiagn. Photodyn., 30 101734 https://doi.org/10.1016/j.pdpdt.2020.101734
(2020).
Google Scholar
W. Feng et al.,
“Quantitative evaluation of skin disorders in type 1 diabetic mice by in vivo optical imaging,”
Biomed. Opt. Express, 10
(6), 2996
–3008 https://doi.org/10.1364/BOE.10.002996 BOEICL 2156-7085
(2019).
Google Scholar
S. M. Zaytsev et al.,
“Optimized skin optical clearing for optical coherence tomography monitoring of encapsulated drug delivery through the hair follicles,”
J. Biophotonics, 13
(4), e201960020 https://doi.org/10.1002/jbio.201960020
(2020).
Google Scholar
H. Zhao et al.,
“A versatile strategy for improving phototherapeutic efficacy on deep-sited tumor by tissue optical clearing technique,”
Nano Today, 36 101058 https://doi.org/10.1016/j.nantod.2020.101058 NTAOCG 1748-0132
(2021).
Google Scholar
C. Liu et al.,
“Quantitative evaluation of enhanced laser tattoo removal by skin optical clearing,”
J. Innov. Opt. Health Sci., 8
(3), 1541007 https://doi.org/10.1142/S1793545815410072
(2015).
Google Scholar
J. Ma et al.,
“Multiple laser pulses in conjunction with an optical clearing agent to improve the curative effect of cutaneous vascular lesions,”
Lasers Med. Sci., 33
(6), 1295
–1306 https://doi.org/10.1007/s10103-018-2481-1
(2018).
Google Scholar
J. Ma et al.,
“Glucose in conjunction with multiple laser pulses on laser treatment of port-wine stain: an in vivo study,”
Lasers Med. Sci., 33
(9), 2015
–2015 https://doi.org/10.1007/s10103-018-2618-2
(2018).
Google Scholar
J. Hirshburg et al.,
“Collagen solubility correlates with skin optical clearing,”
J. Biomed. Opt., 11
(4), 040501 https://doi.org/10.1117/1.2220527 JBOPFO 1083-3668
(2006).
Google Scholar
B. Choi et al.,
“Determination of chemical agent optical clearing potential using in vitro human skin,”
Lasers Surg. Med., 36
(2), 72
–75 https://doi.org/10.1002/lsm.20116 LSMEDI 0196-8092
(2005).
Google Scholar
N. Kuznetsova, S. L. Chi and S. Leikin,
“Sugars and polyols inhibit fibrillogenesis of type I collagen by disrupting hydrogen-bonded water bridges between the helices,”
Biochemistry, 37
(34), 11888
–11895 https://doi.org/10.1021/bi980089+
(1998).
Google Scholar
Z. Mao et al.,
“Influence of alcohols on the optical clearing effect of skin in vitro,”
J. Biomed. Opt., 13
(2), 021104 https://doi.org/10.1117/1.2892684 JBOPFO 1083-3668
(2008).
Google Scholar
J. Hirshburg et al.,
“Correlation between collagen solubility and skin optical clearing using sugars,”
Lasers Surg. Med., 39
(2), 140
–144 https://doi.org/10.1002/lsm.20417 LSMEDI 0196-8092
(2007).
Google Scholar
W. Feng, N. Ma and D. Zhu,
“Molecular effective coverage surface area of optical clearing agents for predicting optical clearing potential,”
Proc. SPIE, 9322 932216 https://doi.org/10.1117/12.2080643
(2015).
Google Scholar
W. Feng et al.,
“Skin optical clearing potential of disaccharides,”
J. Biomed. Opt., 21
(8), 081207 https://doi.org/10.1117/1.JBO.21.8.081207 JBOPFO 1083-3668
(2016).
Google Scholar
J. Wang et al.,
“Sugar-induced skin optical clearing: from molecular dynamics simulation to experimental demonstration,”
IEEE J. Sel. Top. Quantum Electron., 20
(2), 7101007 https://doi.org/10.1109/JSTQE.2013.2289966 IJSQEN 1077-260X
(2014).
Google Scholar
R. Shi et al.,
“FSOCA‐induced switchable footpad skin optical clearing window for blood flow and cell imaging in vivo,”
J. Biophotonics, 10
(12), 1647
–1656 https://doi.org/10.1002/jbio.201700052
(2017).
Google Scholar
J. Wang et al.,
“Ear skin optical clearing for improving blood flow imaging,”
Photonics Lasers Med., 1
(2), 37
–44 https://doi.org/10.1515/plm-2012-0055
(2013).
Google Scholar
J. Wang,
“Optical clearing-induced transparent skin window for improving optical imaging in vivo,”
Huazhong University of Science and Technology, Wuhan, China,
(2013).
Google Scholar
E. A. Genina et al.,
“Integrated effects of fractional laser microablation and sonophoresis on skin immersion optical clearing in vivo,”
J. Biophotonics, 13
(7), e2020000101 https://doi.org/10.1002/jbio.202000101
(2020).
Google Scholar
L. Pires et al.,
“Optical clearing of melanoma in vivo: characterization by diffuse reflectance spectroscopy and optical coherence tomography,”
J. Biomed. Opt., 21
(8), 081210 https://doi.org/10.1117/1.JBO.21.8.081210 JBOPFO 1083-3668
(2016).
Google Scholar
O. Stumpp, B. Chen and A. J. Welch,
“Using sandpaper for noninvasive transepidermal optical skin clearing agent delivery,”
J. Biomed. Opt., 11
(4), 041118 https://doi.org/10.1117/1.2340658 JBOPFO 1083-3668
(2006).
Google Scholar
E. A. Genina et al.,
“Rapid ultrasound optical clearing of human light and dark skin,”
IEEE Trans. Med. Imaging, 39
(10), 3198
–3206 https://doi.org/10.1109/TMI.2020.2989079 ITMID4 0278-0062
(2020).
Google Scholar
Y. Damestani et al.,
“Optical clearing agent perfusion enhancement via combination of microneedle poration, heating and pneumatic pressure,”
Lasers Surg. Med., 46
(6), 488
–498 https://doi.org/10.1002/lsm.22258 LSMEDI 0196-8092
(2014).
Google Scholar
E. A. Genina et al.,
“Optical clearing of human skin: comparative study of permeability and dehydration of intact and photothermally perforated skin,”
J. Biomed. Opt., 13
(2), 021102 https://doi.org/10.1117/1.2899149 JBOPFO 1083-3668
(2008).
Google Scholar
E. A. Genina et al.,
“Optical coherence tomography monitoring of enhanced skin optical clearing in rats in vivo,”
J. Biomed. Opt., 19
(2), 021109 https://doi.org/10.1117/1.JBO.19.2.021109 JBOPFO 1083-3668
(2014).
Google Scholar
R. Shi et al.,
“A useful way to develop effective in vivo skin optical clearing agents,”
J. Biophotonics, 10
(6–7), 887
–895 https://doi.org/10.1002/jbio.201600221
(2017).
Google Scholar
X. Wen et al.,
“Enhanced optical clearing of skin in vivo and optical coherence tomography in-depth imaging,”
J. Biomed. Opt., 17
(6), 066022 https://doi.org/10.1117/1.JBO.17.6.066022 JBOPFO 1083-3668
(2012).
Google Scholar
H. He, Y. Shen and B. Li,
“Rapid skin optical clearing enhancement with salicylic acid for imaging blood vessels in vivo,”
Photodiagn. Photodyn., 32 102005 https://doi.org/10.1016/j.pdpdt.2020.102005
(2020).
Google Scholar
H. Shan et al.,
“Study on application of optical clearing technique in skin diseases,”
J. Biomed. Opt., 17
(11), 115003 https://doi.org/10.1117/1.JBO.17.11.115003 JBOPFO 1083-3668
(2012).
Google Scholar
M. P. Ouyang et al.,
“Skin optical clearing enabled by dissolving hyaluronic acid microneedle patches,”
Int. J. Biol. Macromol., 220 1188
–1196 https://doi.org/10.1016/j.ijbiomac.2022.08.153 IJBMDR 0141-8130
(2022).
Google Scholar
D. K. Tuchina et al.,
“Magnetic resonance contrast agents in optical clearing: prospects for multimodal tissue imaging,”
J. Biophotonics, 13
(11), e201960249 https://doi.org/10.1002/jbio.201960249
(2020).
Google Scholar
N. I. Kazachkina et al.,
“MR and fluorescence imaging of gadobutrol-induced optical clearing of red fluorescent protein signal in an in vivo cancer model,”
NMR Biomed., 35
(7), e4708 https://doi.org/10.1002/nbm.4708
(2022).
Google Scholar
A. Sdobnov et al.,
“A comparative study of ex vivo skin optical clearing using two-photon microscopy,”
J. Biophotonics, 10
(9), 1115
–1123 https://doi.org/10.1002/jbio.201600066
(2017).
Google Scholar
A. Y. Sdobnov et al.,
“Confocal Raman microscopy supported by optical clearing treatment of the skin-influence on collagen hydration,”
J. Phys. D Appl. Phys., 50
(28), 285401 https://doi.org/10.1088/1361-6463/aa77c9
(2017).
Google Scholar
X. Liu and B. Chen,
“In vivo experimental study on the enhancement of optical clearing effect by laser irradiation in conjunction with a chemical penetration enhancer,”
Appl. Sci.-Basel, 9
(3), 542 https://doi.org/10.3390/app9030542
(2019).
Google Scholar
J. L. M. Hawk,
“Safe, mild ultraviolet-B exposure: an essential human requirement for vitamin D and other vital bodily parameter adequacy: a review,”
Photodermatol. Photoimmunol. Photomed., 36
(6), 417
–423 https://doi.org/10.1111/phpp.12584 PPPHEW 0905-4383
(2020).
Google Scholar
L. Kemeny, E. Varga and Z. Novak,
“Advances in phototherapy for psoriasis and atopic dermatitis,”
Expert Rev. Clin. Immunol., 15
(11), 1205
–1214 https://doi.org/10.1080/1744666X.2020.1672537
(2019).
Google Scholar
J. Ye et al.,
“NB-UVB irradiation attenuates inflammatory response in psoriasis,”
Dermatol. Ther., 33
(4), e13626 https://doi.org/10.1111/dth.13626
(2020).
Google Scholar
X. Yang et al.,
“Biomedical applications of terahertz spectroscopy and imaging,”
Trends Biotechnol., 34
(10), 810
–824 https://doi.org/10.1016/j.tibtech.2016.04.008 TRBIDM 0167-7799
(2016).
Google Scholar
I. Carneiro et al.,
“Moving tissue spectral window to the deep-ultraviolet via optical clearing,”
J. Biophotonics, 12
(12), e201900181 https://doi.org/10.1002/jbio.201900181
(2019).
Google Scholar
I. Carneiro et al.,
“Enhanced ultraviolet spectroscopy by optical clearing for biomedical applications,”
IEEE J. Sel. Top. Quantum Electron., 27
(4), 7200108
–8 https://doi.org/10.1109/JSTQE.2020.3012350 IJSQEN 1077-260X
(2021).
Google Scholar
A. A. Selifonov and V. V. Tuchin,
“Tissue optical clearing in the ultraviolet for clinical use in dentistry to optimize the treatment of chronic recurrent aphthous stomatitis,”
J. Biomed. Photonics Eng., 6
(4), 040301 https://doi.org/10.18287/JBPE20.06.040301
(2020).
Google Scholar
A. Selifonov, E. Selifonova and V. V. Tuchin,
“Effect of e-liquid on the optical properties of the gingival mucosa: ex vivo studies,”
IEEE J. Sel. Top. Quantum Electron., 29
(4), 1
–8 https://doi.org/10.1109/JSTQE.2023.3259244 IJSQEN 1077-260X
(2023).
Google Scholar
A. S. Kolesnikov et al.,
“THz monitoring of the dehydration of biological tissues affected by hyperosmotic agents,”
Phys. Wave Phenom., 22
(3), 169
–176 https://doi.org/10.3103/S1541308X14030029
(2014).
Google Scholar
G. R. Musina et al.,
“Optimal hyperosmotic agents for tissue immersion optical clearing in terahertz biophotonics,”
J. Biophotonics, 13
(12), e202000297 https://doi.org/10.1002/jbio.202000297
(2020).
Google Scholar
S. J. Oh et al.,
“Measurement depth enhancement in terahertz imaging of biological tissues,”
Opt. Express, 21
(18), 21299
–21305 https://doi.org/10.1364/OE.21.021299 OPEXFF 1094-4087
(2013).
Google Scholar
W. Feng et al.,
“Visualization of skin microvascular dysfunction of type 1 diabetic mice using in vivo skin optical clearing method,”
J. Biomed. Opt., 24
(3), 031003 https://doi.org/10.1117/1.JBO.24.3.031003 JBOPFO 1083-3668
(2019).
Google Scholar
C. Zhang and W. Feng,
“Assessment of tissue-specific changes in structure and function induced by in vivo skin/skull optical clearing techniques,”
Lasers Surg. Med., 54
(3), 447
–458 https://doi.org/10.1002/lsm.23489 LSMEDI 0196-8092
(2022).
Google Scholar
M. V. Novoselova et al.,
“Optical clearing for photoacoustic lympho- and angiography beyond conventional depth limit in vivo,”
Photoacoustics, 20 100186 https://doi.org/10.1016/j.pacs.2020.100186
(2020).
Google Scholar
W. Feng, C. Zhang and D. Zhu,
“Comparison of cortical and cutaneous vascular hemodynamic changes in hypoxia by using in vivo skull and skin optical clearing techniques,”
IEEE J. Sel. Top. Quantum Electron., 27
(4), 1
–7 https://doi.org/10.1109/JSTQE.2021.3062595 IJSQEN 1077-260X
(2021).
Google Scholar
L. Guo et al.,
“Optical coherence tomography angiography offers comprehensive evaluation of skin optical clearing in vivo by quantifying optical properties and blood flow imaging simultaneously,”
J. Biomed. Opt., 21
(8), 081202 https://doi.org/10.1117/1.JBO.21.8.081202 JBOPFO 1083-3668
(2016).
Google Scholar
A. Banerjee and R. Poddar,
“Enhanced visualization of tissue microstructures using swept-source optical coherence tomography and edible oil as optical clearing agent,”
Optik, 267 169693 https://doi.org/10.1016/j.ijleo.2022.169693 OTIKAJ 0030-4026
(2022).
Google Scholar
A. Jaafar et al.,
“Ex-vivo confocal Raman microspectroscopy of porcine skin with 633/785-NM laser excitation and optical clearing with glycerol/water/DMSO solution,”
J. Innov. Opt. Health Sci., 14
(5), 2142003 https://doi.org/10.1142/S1793545821420037
(2021).
Google Scholar
B. Khlebtsov et al.,
“Improving SERS bioimaging of subcutaneous phantom in vivo with optical clearing,”
J. Biophotonics, 15
(3), e202100281 https://doi.org/10.1002/jbio.202100281
(2022).
Google Scholar
Y. Zhan et al.,
“Noninvasively imaging subcutaneous tumor xenograft by a handheld raman detector, with the assistance of an optical clearing agent,”
ACS Appl. Mater. Interfaces, 9
(21), 17769
–17776 https://doi.org/10.1021/acsami.7b04205 AAMICK 1944-8244
(2017).
Google Scholar
Y. A. Menyaev et al.,
“Optical clearing in photoacoustic flow cytometry,”
Biomed. Opt. Express, 4
(12), 3030
–3041 https://doi.org/10.1364/BOE.4.003030 BOEICL 2156-7085
(2013).
Google Scholar
Y. Ding et al.,
“Signal and depth enhancement for in vivo flow cytometer measurement of ear skin by optical clearing agents,”
Biomed. Opt. Express, 4
(11), 2518
–2526 https://doi.org/10.1364/BOE.4.002518 BOEICL 2156-7085
(2013).
Google Scholar
Y. Liu et al.,
“Optical clearing agents improve photoacoustic imaging in the optical diffusive regime,”
Opt. Lett., 38
(20), 4236
–4239 https://doi.org/10.1364/OL.38.004236 OPLEDP 0146-9592
(2013).
Google Scholar
J. Pan et al.,
“A miniaturized fluorescence imaging device for rapid early skin cancer detection,”
J. Innov. Opt. Health Sci., 14
(2), 2050026 https://doi.org/10.1142/S1793545820500261
(2021).
Google Scholar
J. K. Chen et al.,
“An overview of clinical and experimental treatment modalities for port wine stains,”
J. Am. Acad. Dermatol., 67
(2), 289
–304.e29 https://doi.org/10.1016/j.jaad.2011.11.938 JAADDB 0190-9622
(2012).
Google Scholar
Y. Zhu et al.,
“Real-time vascular and IgA dynamics during Henoch-Schonlein purpura by in vivo fluorescent microscopy,”
Biomed. Opt. Express, 12
(12), 7826
–7834 https://doi.org/10.1364/BOE.442454 BOEICL 2156-7085
(2021).
Google Scholar
Y. Lu et al.,
“An optical system for noninvasive microscopy of psoriatic mice in vivo,”
J. Biophotonics, 16
(4), e202200310 https://doi.org/10.1002/jbio.202200310
(2023).
Google Scholar
J. Zhu et al.,
“Optical angiography for diabetes-induced pathological changes in microvascular structure and function: an overview,”
J. Innov. Opt. Health Sci., 15
(01), 2230002 https://doi.org/10.1142/S1793545822300026
(2022).
Google Scholar
R. Shi et al.,
“In vivo imaging the motility of monocyte/macrophage during inflammation in diabetic mice,”
J. Biophotonics, 11
(5), e201700205 https://doi.org/10.1002/jbio.201700205
(2018).
Google Scholar
W. Feng et al.,
“Comparison of cerebral and cutaneous microvascular dysfunction with the development of type 1 diabetes,”
Theranostics, 9
(20), 5854
–5868 https://doi.org/10.7150/thno.33738
(2019).
Google Scholar
D. K. Tuchina et al.,
“Ex vivo optical measurements of glucose diffusion kinetics in native and diabetic mouse skin,”
J. Biophotonics, 8
(4), 332
–346 https://doi.org/10.1002/jbio.201400138
(2015).
Google Scholar
D. Tuchina et al.,
“Study of glycerol diffusion in skin and myocardium ex vivo under the conditions of developing alloxan-induced diabetes,”
J. Biomed. Photonics Eng., 3
(2), 020302 https://doi.org/10.18287/JBPE17.03.020302
(2017).
Google Scholar
X. Li et al.,
“Sensitive and high resolution subcutaneous fluorescence in vivo imaging using upconversion nanoparticles and microarrays,”
Analyst, 138
(13), 3711
–3718 https://doi.org/10.1039/c3an00441d ANLYAG 0365-4885
(2013).
Google Scholar
H. Arora et al.,
“Lasers for nevi: a review,”
Lasers Med. Sci., 30
(7), 1991
–2001 https://doi.org/10.1007/s10103-014-1697-y
(2015).
Google Scholar
A. Klein et al.,
“Laser and IPL treatment of port-wine stains: therapy options, limitations, and practical aspects,”
Lasers Med. Sci., 26
(6), 845
–859 https://doi.org/10.1007/s10103-011-0903-4
(2011).
Google Scholar
G. R. Schweinsberger et al.,
“Noninvasive laser coagulation of the human vas deferens: optical and thermal simulations,”
Lasers Surg. Med., 43
(5), 443
–449 https://doi.org/10.1002/lsm.21057 LSMEDI 0196-8092
(2011).
Google Scholar
R. G. Geronemus,
“Long-pulsed neodymium: yttrium-aluminum-garnet laser treatment for port wine stains,”
J. Am. Acad. Dermatol., 54
(5), 923
–923 https://doi.org/10.1016/j.jaad.2005.07.031 JAADDB 0190-9622
(2006).
Google Scholar
W. Jia et al.,
“Photocoagulation of dermal blood vessels with multiple laser pulses in an in vivo microvascular model,”
Lasers Surg. Med., 44
(2), 144
–151 https://doi.org/10.1002/lsm.22000 LSMEDI 0196-8092
(2012).
Google Scholar
C. M. Cilip et al.,
“Application of an optical clearing agent during noninvasive laser coagulation of the canine vas deferens,”
J. Biomed. Opt., 15
(4), 048001 https://doi.org/10.1117/1.3463009 JBOPFO 1083-3668
(2010).
Google Scholar
V. D. Genin et al,
“Influence of immersion agents on optical parameters of bio-tissues during laser photothermal therapy of tumor: pilot study,”
Opt. Spectrosc., 130
(6), 678
–687 https://doi.org/10.21883/EOS.2022.06.54704.27-22 OPSUA3 0030-400X
(2022).
Google Scholar
L. Pires et al.,
“Dual-agent photodynamic therapy with optical clearing eradicates pigmented melanoma in preclinical tumor models,”
Cancers, 12
(7), 1956 https://doi.org/10.3390/cancers12071956
(2020).
Google Scholar
Y. Chu et al.,
“Second near-infrared photothermal therapy with superior penetrability through skin tissues,”
CCS Chem., 4
(9), 3002
–3013 https://doi.org/10.31635/ccschem.021.202101539
(2022).
Google Scholar
H. Yu et al.,
“Collaborative effects of wavefront shaping and optical clearing agent in optical coherence tomography,”
J. Biomed. Opt., 21
(12), 121510 https://doi.org/10.1117/1.JBO.21.12.121510 JBOPFO 1083-3668
(2016).
Google Scholar
D. Li et al.,
“Transmissive-detected laser speckle contrast imaging for blood flow monitoring in thick tissue: from Monte Carlo simulation to experimental demonstration,”
Light Sci. Appl., 10
(1), 241 https://doi.org/10.1038/s41377-021-00682-8
(2021).
Google Scholar
X. Sang, D. Li and B. Chen,
“Improving imaging depth by dynamic laser speckle imaging and topical optical clearing for in vivo blood flow monitoring,”
Lasers Med. Sci., 36
(2), 387
–399 https://doi.org/10.1007/s10103-020-03059-2
(2021).
Google Scholar
J. C. Ramirez-San-Juan et al.,
“Spatial versus temporal laser speckle contrast analyses in the presence of static optical scatterers,”
J. Biomed. Opt., 19
(10), 106009 https://doi.org/10.1117/1.JBO.19.10.106009 JBOPFO 1083-3668
(2014).
Google Scholar
BiographyQing Xia is a PhD candidate student of the Wuhan National Laboratory for Optoelectronics, Huazhong University of Science and Technology (HUST), China. She received her bachelor’s degree from the School of Optoelectronic Engineering, Changchun University of Science and Technology, China, in 2019. Her current research fields lie in biomedical photonics. Dongyu Li received his PhD from the College of Optical Science and Engineering of Zhejiang University, China, in 2019. He is now an associate professor in the School of Optical Electronic Information, HUST, China. His research work focuses on biomedical photonics. He is the first author or corresponding author of more than 20 peer-reviewed SCI papers, such as advanced materials, light: science and applications, etc. Tingting Yu received her PhD in biomedical photonics from HUST, China, in 2015. She is now an associate professor at Wuhan National Laboratory for Optoelectronics, HUST. Her research work focuses on tissue optical clearing methods and applications. Jingtan Zhu received his PhD from Wuhan National Laboratory for Optoelectronics, HUST, China, in 2020. He is now a postdoctoral researcher at Wuhan National Laboratory for Optoelectronics, HUST. His research work focuses on biomedical photonics, especially for tissue optical clearing and imaging. He has published several SCI-indexed papers in international journals, such as Advanced Science and Science Advances. Dan Zhu is a Distinguished Professor at HUST, Director of the Advanced Biomedical Imaging Facility, and Vice-Director with Wuhan National Laboratory for Optoelectronics. Her research interest focuses on tissue optical clearing imaging. She has authored more than 200 papers. She serves as an associate editor, editorial board member, or guest editor for international journals. She is a Fellow of SPIE and the Secretary General & Vice-President of the Biomedical Photonics Committee of the Chinese Optical Society. |
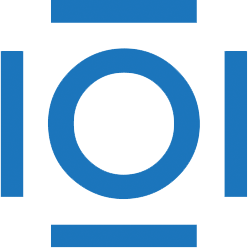
CITATIONS
Cited by 3 scholarly publications.
Skin
Optical clearing
In vivo imaging
Optical imaging
Tissues
Tumors
Biomedical optics