1.IntroductionInvestigation of the spatiotemporal characteristics of blood flow in tissue is important for understanding the physiology and pathology of tissues of interest. For this purpose, various techniques for two-dimensional blood flow imaging have been suggested. One of these is laser Doppler perfusion imaging (LDPI),1 2 3 which is a rather new method for visualization of blood flow over a large area of tissue. In these systems, the tissue is illuminated by a narrow, collimated laser beam, and a detector at some distance from the tissue is used to observe the light reemitted from the illuminated area. The beam is scanned through a certain tissue area, yielding a 2-D perfusion image, defined as the average blood cell velocity multiplied by the blood cell concentration in the measured volume. However, in this method the temporal and spatial resolution is limited by the need for scanning. Konishi and Fujii et al.;4 developed a technique for mapping retinal blood flow by use of a 2-D CCD array without a scanning mirror, which produced a microcirculation map of the retina by measuring the blur rate of the detected interferometric pattern. However, the integration of the signal variation in the sensor in particular made quantitative estimation of the flow speed difficult, since the blur rate of the analyzed parameter was also dependent on other factors, such as capillary diameter, density of the moving scatterers, and the scattering properties of the background tissue. An alternative full-field real-time technique, laser speckle contrast analysis (LASCA), which is based on the first-order spatial statistics of time-integrated speckle, was suggested by Briers et al.;5 A 2-D array CCD camera with focusing optics was used to detect the speckle pattern formed by light reemitted from tissue illuminated by a divergent laser beam. Analyses of the speckle pattern contrast provided information about the concentration and average velocity of red blood cells.6 Recently Dunn7 and Bolay et al.;8 successfully used this method to image cerebral blood flow changes during focal cerebral ischemia and cortical spreading depression. The main disadvantage of LASCA is the loss of resolution caused by the need to average over a block of pixels to obtain the spatial statistics used in the analysis, although it actually has higher resolution than other techniques, such as scanning laser Doppler. Recently, a laser Doppler perfusion imaging method with a complementary metal oxide semiconductor sensor was proposed by Serov et al.;,6 which could provide higher temporal resolution than that of existing mechanical laser Doppler perfusion imagers, since no integration time is involved. However, in this method, an improvement is needed to change the system into a compact, easy-to-use instrument, and it is difficult to obtain a full-frame mode image of the areas of interest for a long measurement time because of the large amount of data that has to be processed. In this paper we present a modified laser speckle imaging (LSI) method that uses the temporal statistics of the time-integrated speckle based on our previous studies.9 10 First a model experiment was designed to validate this method, and then imaging of the distribution of rat cerebral blood flow (CBF) was performed. The influence of temperature on rat cerebral blood flow was also investigated by this method and compared with the results from LASCA. 2.Materials and Methods2.1.LSI SystemFigure 1 was a schematic diagram of the experimental setup. A helium:neon (He:Ne) laser beam (λ=632.8 nm, 3 MW) was coupled into an 8-mm diameter fiber bundle, which was adjusted to illuminate the area of interest evenly. The illuminated area was imaged through a zoom stereo microscope (SZ6045TR, Olympus, Japan) onto a CCD camera (PIXELFLY, PCO Computer Optics, Germany) with 480×640 pixels, yielding an image of 0.8 to 7 mm depending on the magnification. The exposure time T of the CCD was 20 ms. Images were acquired through easy-control software (PCO Computer Optics, Germany) at 40 Hz. 2.2.Model ExperimentA porcelain plane, which was pushed by a stepping motor (SC3, Sinoptek, China), moved with a velocity that ranged from 0.018 to 2.3 mm/s. The laser beam illuminated the surface of the plane evenly and the imaged area was about 2.46×3.28 mm. Three measurements were performed under each velocity condition. 2.3.In Vivo CBF MeasurementThe experiment was performed on Sprague-Dawley rats (350 to 450 g), which were anesthetized with α-chloralose and urethane (50 and 600 mg/kg, respectively). The right femoral artery was cannulated for measurement of mean arterial blood pressure (Pclab Instruments, China) and blood sampling. A tracheotomy was executed to enable mechanical ventilation with a mixture of air and oxygen (20 O 2, 80 N 2, TKR-200C, China). Periodically, blood gases were analyzed to ensure that noemoxia was maintained at normal physiological levels (Pa O 2 , Pa CO 2 , and pH) (JBP-607, Dissolved Oxygen Analyzer, China). The animals were mounted in a stereotaxic frame, and rectal temperature was maintained at 37.0±0.5°C with a thermostatic heating blanket. The skull was thinned to translucency using a dental drill under constant cooling with saline. Following surgical preparation, the animals were left for at least 30 min before the experiment began. In all animals, the physiological parameters were kept within normal range throughout the experiments. The temperature of the rat cortex was changed locally by constant application of warm saline solution to the cortex for 10 min under each temperature condition: 35, 45, and 50 °C. The raw speckle images were acquired first under the control condition (38 °C), then under other temperatures to obtain the CBF map. 2.4.Speckle Image ProcessingLaser speckle is an interference pattern produced by the light reflected or scattered from different parts of an illuminated rough (i.e., nonspecular) surface. When the area illuminated by a laser light is imaged onto a camera, a granular or speckle pattern is produce. If the scattered particles are moving, a time-varying speckle pattern is generated at each pixel in the image. The intensity variations of this pattern contain information on the scattered particles. 2.4.1.Analysis of LASCAIn the current version of LASCA,5 to quantify the blurring of the speckles, the local speckle contrast is defined as the ratio of the standard deviation to the mean intensity in a small region of the image: where k, σs, and 〈I〉 represent speckle contrast, the standard deviation, and the mean value of light intensity, respectively. This method uses the spatial intensity variations in a speckle pattern to obtain the relative blood flow map. In practice, a 5×5 or 7×7 region of pixels is used. Lower numbers reduce the validity of the statistics, whereas higher numbers limit the spatial resolution of the technique. In this study, squares of 5×5 pixels were used. The software computes the speckle contrast k for any given square and assigns this value to the central pixel of the square. The process is then repeated for 5×5 squares centered on each pixel in turn. This results in a smoothing of the contrast map, but the spatial resolution is lost in averaging over a block of pixels.2.4.2.Theory and analysis of modified LSIThe first-order temporal statistics of time-integrated speckle patterns can be used to obtain velocity information; these were described in detail in Ref. 11. In the previous research, only the velocity of a single point area (a single speckle size in the detected plane) was measured by this method. where 〈I〉 and 〈I2〉 are the mean and mean-square values of time-integrated speckle intensity variations during the time interval t and Nt is inversely proportional to the velocity of the scattering particle.11Here we utilized the first-order temporal statistics of time-integrated speckle to obtain a 2-D distribution of blood flow. Each pixel in the speckle image can be viewed as the single point area in the previous study. Then the signal processing consists of calculating the temporal statistics of the intensity of each pixel in the image: where Ii,j,t is the instantaneous intensity of the i’ and j’th pixels at the t’th frame of raw speckle images, and 〈Ii,j,t〉t is the average intensity of the i’ and j’th pixels over the consecutive m frames. Ni,j is inversely proportional to the velocity of the scattering particles. The value Ni,j of each pixel in the consecutive m frames (Ii,j,t) of the raw speckle pattern is computed according to Eq. (2). The process is then repeated for the next group of m frames. The results are given as 2-D gray-scale (65,536 shades) or false-color (65,536 colors) coded maps that describe the spatial variation of the velocity distribution in the area examined.3.Results and Discussion3.1.Validation of the Modified LSI MethodIn the model experiment, the N values of the center pixel of the area of interest under different velocities were computed according to Eq. (2). Figure 2 shows the value of the reciprocal of N(1/N) computed from a different number of frames (m) of consecutive images under different velocity conditions (V). The correlation value (R2) between V and 1/N is given in Fig. 3. It is obvious that the correlation value increased with m. When m was larger than 15, a high correlation (R2=0.96) was obtained. The reason is that for a small number of integrated speckles, the experimental results of the probability density function are slightly different from the theoretical ones (gamma distribution), which may be due to the statistical uncertainty associated with the experiments,11 and therefore the linearity is only for high m. The linearity is not as good as that for LDPI, for Eq. (2) is obtained under an ideal condition of a fully developed speckle pattern,5 11 and actual experimental conditions usually only approximate the ideal ones, which does not have much effect in measuring the relative velocity change. In Fig. 2, the high 1/N values obtained for the lower m may be due to the fact that for a small number of integrated speckles, the fluctuation of the moving porcelain plane becomes significant and its effect cannot be neglected,11 i.e., for a lower m, the value of [〈I2〉−〈I〉2]1/2/〈I〉 (between 0 and 1, reflecting the fluctuation) would be larger and N would be smaller, and thus the corresponding 1/N value becomes larger. To ensure the temporal resolution, we could choose an m larger than 15. Assuming ergodicity, the principle of the modified LSI method was similar to that of LASCA. In theory, to obtain the same the signal-to-noise ratio (SNR) as LASCA, twenty-five temporal samples must be used, i.e., m equals 25. Here we compare the blood flow map obtained when m equaled 25 with that obtained by LASCA. The least-squares fit between V and 1/N was displayed when m equaled 25 (Fig. 2), which suggested that the change of the 1/N value could be used to effectively reflect the velocity change of scatterers in the illuminated area. The same problem of the difficultly of measuring the absolute velocity of scatterers from the time-integrated speckle pattern was encountered; this is a problem shared with all time-varying speckle techniques, as well as with laser Doppler.5 12 13 Figure 2The value of 1/N under different velocity (V) conditions calculated through different frames. The solid line is a least-squares fit between 1/N and V when m equals 25. ![]() As stated in previous studies of image speckle,5 7 14 each point in the image plane is the superimposed result of the points near the corresponding point in the object plane, i.e., the size of a single speckle was approximately equal to the size of a single pixel in the image acquired by CCD, and the photos from different speckles did not interact with others, which was different from the case of laser Doppler imaging. Each 1/Ni,j value reflected the velocity change for one pixel (i,j) in the imaged area. If the velocities of the imaged plane were diverse, like the inhomogeneities in the CBF model, the N value of each pixel would be different, forming the velocity map of CBF. We chose a moving porcelain plane as our model for the convenience of controlling the speed by computer. Of course, a tube model using a layer of static scatter above with different velocities and concentrations of Intralipid (or blood) would be better than this model because of its close resemblance to a real CBF model. Further research is needed to clarify the relationship between the signal and the velocity and concentration by a tube model. 3.2.CBF Maps Obtained by Modified LSIA white-light image of the rat cerebral vasculature is shown in Fig. 4(a). By focusing the expanded laser beam on the areas of interest (2.46×3.28 mm), raw speckle images were obtained. The CBF maps obtained by our modified LSI method are shown in Figs. 4(b), 4(c), 4(d), and 4(e). These were the blood flow maps obtained with 5, 8, 15, and 25 frames (m), respectively. It is easy to see that as m increased, the signal-to-noise ratio in the blood flow map increased. Figure 4Blood flow maps obtained by the modified LSI and LASCA under the control condition. (a) A white-light picture of rat brain under the control condition. (b), (c), (d), and (e) Blood flow maps obtained by our modified method when m was chosen as 5, 8, 15, and 25 (color bar indicates N value). (f) Blood flow map obtained by LASCA (color bar indicates K value). Scale bar: 500 μm. ![]() 3.3.Spatial Resolution of the Modified LSIIn our in vivo CBF experiment, the field of view was approximately 2.46×3.28 mm. The size of each raw speckle image was 480×640 pixels. According to the principle of the modified LSI, the resolution of the map was 5 μm (246/480). On the other hand, the resolution of the map obtained by LASCA was effectively reduced by the use of 5×5 squares of pixels from 480×640 (pixels) to approximately 96×128 (pixel blocks).5 Hence the spatial resolution was approximately 25 μm, which is one-fifth that of the modified LSI in theory. In a comparison of our results with the work by Linden et al.;3 in which an enhanced high-resolution laser Doppler imaging (EHR-LDI) technique intended for the visualization of separate microvessels was evaluated by use of in vitro flow models, the resolution of our modified LSI was much higher than that of EHR-LDI (about 40 μm). The modified LSI and LASCA were utilized to measure the CBF under the same conditions. According to Eq. (2), maps of flows represented by N values under the control condition (38 °C) were obtained [Fig. 4(e), m=25] . In comparing our map with the map obtained by LASCA [Fig. 4(f)], we could see that the spatial resolution of our modified method was much higher; more small blood vessels appeared clearly in the case of the modified LSI, although both methods could well resolve the statically and dynamically scattering regions in the flow map. 3.4.Influence of Temperature on CBFThe CBF distributions of the rats at different temperatures (35, 45, and 50 °C) were examined in this study. The results are illustrated in Fig. 5. When the temperature was increased from 35 to 50 °C, the flow map obtained by the modified LSI became darker [Figs. 5(a), 5(b), and 5(c)] indicating that the N value became smaller; therefore the blood perfusion increased. In some small blood vessels, indicated by panes in Fig. 5(a), blood perfusion obviously increased. However, these vessels could not be clearly seen in the map obtained by LASCA [Figs. 5(d), 5(e), and 5(f)] owing to the lower spatial resolution, although these two methods showed the same tendency of a thermal influence on CBF. Hence, in physiological studies, the modified LSI could provide more information about small blood vessels. Since brain homeostasis depends on adequate levels of blood flow to ensure the delivery of nutrients and to facilitate the removal of metabolites and excess heat, the exchange of material between constituents in the blood and the neurons and glia occurs at the level of individual capillaries.15 Our modified LSI with improved spatial resolution would be helpful for these brain researches. Figure 5Blood flow maps obtained by two methods. (a), (b), and (c) Blood flow distribution obtained by the modified LSI when m equaled 25 at temperatures of 35, 45, and 50 °C, respectively (the color bar indicates N values). (d), (e), and (f) Blood flow distribution obtained by LASCA at temperatures of 35, 45, and 50 °C, respectively (the color bar indicates K values). The outlined areas in (a) indicate a change in the blood perfusion of small vessels in response to temperature. The darker areas in the maps obtained by the two methods both show increased cerebral blood flow. Scale bar: 500 μm. ![]() 3.5.Temporal Resolution of the Modified LSIThe sampling frame of our CCD was 40 Hz. Using the modified LSI method, the temporal resolution was about 0.4 to 0.6 s (15/40, 25/40), which was determined by the sampling frequency of the CCD camera and the value of m. As described earlier, the temporal resolution of LASCA was only determined by the sampling frequency (1/40 s), which was higher than that of the modified LSI. In many cases of physiological studies, the CBF response is a slow change,6 7 and the temporal resolution of seconds was enough for a measurement. On the other hand, the data processing time of this method was reduced a lot, which is an advantage in a real-time operation. 4.ConclusionIn contrast to other LDPI methods, our modified laser speckle imaging method does not need moving scanning components, and the spatial resolution is five times higher than that of LASCA. Thus the LSI is able to distinguish small blood vessels and provide more spatial information under the same conditions. The temporal resolution of this method, however, is much lower than that of LASCA and of laser Doppler perfusion imaging with a complementary metal oxide semiconductor sensor that was recently proposed by Serov et al.;8 because of the limitation of the frame rate of the CCD camera. However, this method could be used to measure the relatively slow change of blood flow. Using a CCD camera with a high sampling frame rate would increase the temporal resolution. Also, our system is an easy-to-use instrument for the whole field of blood flow imaging. AcknowledgmentsThis work was supported by the National Nature Science Foundation of China (NSFC) (grants 59836240, 30070215, 30170306, 60178028), and the NSFC for distinguished young scholars (grant 60025514). REFERENCES
B. M. Ances
,
J. H. Greenberg
, and
J. A. Detre
,
“Laser Doppler imaging of activation-flow coupling in the rat somatosensory cortex,”
Neuroimage , 10 716
–723
(1999). Google Scholar
T. J. H. Essex
and
P. O. Byrne
,
“A laser Doppler scanner for imaging blood flow in skin,”
J. Biomed. Eng. , 13 189
–194
(1991). Google Scholar
M. Linden
,
H. Golster
,
S. Bertuglia
,
A. Colantuoni
,
F. Sjoberg
, and
G. Nilsson
,
“Evaluation of enhanced high-resolution laser Doppler imaging in an in vitro tube model with the aim of assessing blood flow in a separate microvessel,”
Microvasc. Res. , 56 261
–270
(1998). Google Scholar
N. Konishi
and
H. Fujii
,
“Real-time visualization of retinal microcirculation by laser flowgraphy,”
Opt. Eng. , 34 753
–757
(1995). Google Scholar
J. D. Briers
and
S. Webster
,
“Laser speckle contrast analysis (LASCA): a non-scanning, full-field technique for monitoring capillary blood flow,”
J. Biomed. Opt. , 1 174
–179
(1996). Google Scholar
A. Serov
,
W. Steenbergen
, and
F. D. Mul
,
“Laser Doppler perfusion with a complementary metal oxide semiconductor image sensor,”
Opt. Lett. , 27 300
–302
(2002). Google Scholar
A. K. Dunn
,
H. Bolay
,
M. A. Moskowitz
, and
A. B. David
,
“Dynamic imaging of cerebral blood flow using laser speckle,”
J. Cereb. Blood Flow Metab. , 21 195
–201
(2001). Google Scholar
H. Bolay
,
U. Reuter
,
A. K. Dunn
,
Z. Huang
,
D. A. Boas
, and
A. M. Moskowitz
,
“Intrinsic brain activity triggers trigeminal meningeal affererents in a migraine model,”
Nat. Med. , 8 136
–142
(2002). Google Scholar
H. Cheng
,
Q. Luo
,
S. Zeng
,
J. Cen
, and
W. Liang
,
“Optical dynamic imaging of the regional blood flow in the rat mesentery under the drug’s effect,”
Prog. Nat. Sci. , 13 397
–400
(2003). Google Scholar
J. Ohtsubo
and
T. Asakura
,
“Velocity measurement of a diffuse object by using time-varying speckles,”
Opt. Quant. Electron. , 8 523
–529
(1976). Google Scholar
R. Bonner
and
R. Nossal
,
“Model for laser Doppler measurements of blood flow in tissue,”
Appl. Opt. , 20 2097
–2107
(1981). Google Scholar
J. D. Briers
,
“Laser Doppler and time-varying speckle: reconciliation,”
J. Opt. Soc. Am. A , 13 345
–350
(1996). Google Scholar
D. Kleinfeld
,
P. P. Mitra
,
F. Helmchen
, and
W. Denk
,
“Fluctuations and stimulus-induced changes in blood flow observed in individual capillaries in layers 2 through 4 of rat neocortex,”
Proc. Natl. Acad. Sci. U.S.A. , 95 15741
–15746
(1998). Google Scholar
|
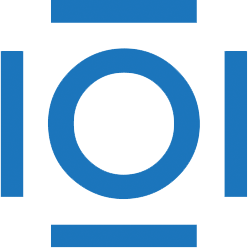
CITATIONS
Cited by 226 scholarly publications and 5 patents.
Blood circulation
Speckle
Spatial resolution
Blood
Speckle pattern
Laser speckle imaging
Doppler effect